Cell Culture - An Introduction
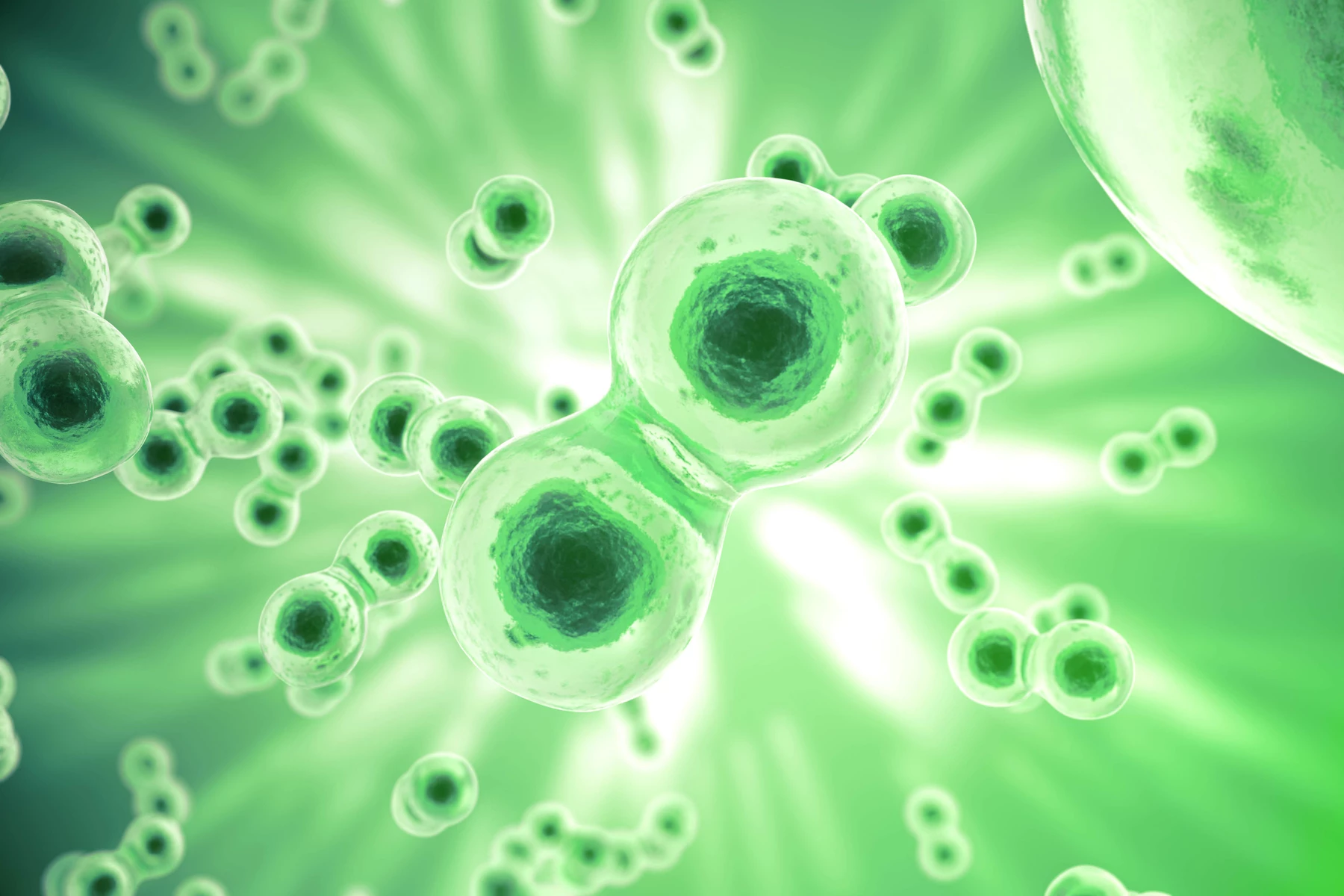
Cell Culture - An Introduction
Cells, the fundamental unit of life, are an incredibly useful tool in biological research. They serve as an imperative model system for understanding physiological processes and screening of toxic or therapeutic compounds for use in medical treatments. In addition, cells play a major role in functional enzyme, growth factor and vaccine production, along with a multitude of other uses. In order to accomplish aforementioned practices, cells must first be cultured in an environment outside from the organism they originate. This is the process of cell culture. To elaborate, cell culturing involves maintaining cells of multi-cellular organisms outside of their original body under precise conditions (1).
Contents
- Cell Culture – An Introduction
Cell Culture in Research Areas
Cell Culture In Vaccine Research
Cell culture has a diverse range of uses as cultured cells are used by cell biologists, biomaterials scientists, clinicians and regulatory authorities, among others(2). One of the most important uses of cell culture is in the research and production of vaccines. The ability to grow large amounts of virus in cell culture eventually led to the creation of the polio vaccine, and cells are still used today on a large scale to produce vaccines for many other diseases. Early in the 1930s and 40s, researchers had to use live animals to grow poliovirus, but with the advent of cell culture, they were able to achieve much greater control over virus production and on a much larger scale (3). This allowed researchers to study them more closely and eventually develop vaccines and various treatments. In immunotherapy, cell culture in vaccine research against cancer.
Cell Culture In Protein Therapeutics
Another important use of cell lines is to express different types of proteins in mammalian cells (2). Initially, E. coli was the primary organism used for producing proteins, but with the need to create properly folded proteins with the proper post-translational modifications, the focus shifted to using eukaryotes instead (4). Starting from the 1970s and 80s, proteins like interferon and antibodies have been successfully created via cell culture. Various cytokines and growth factors can be acquired from cultures, and looking at the structure and activity of these proteins helps us understand their role in the organism’s body as well. Even observing the protein grown in different cells and conditions may help us determine how the protein’s environment affects its activity.
Cell culture In Cancer Research
Cancer is one of the leading causes of death around the world and millions of dollars are being used for cancer research to find treatments and cures. Cell culture is crucial here as well, as normal cells can be transformed into cancer cells by methods including radiation, chemicals and viruses (5). These cells can then be used to study cancer more closely and to test potential new treatments.
Primary Cells versus Cell Lines
Primary cells and established cell lines are most used cells in the lab.
The Primary cells, also known as finite cells, are cells directly prepared from an organism’s tissues (6). If grown under the right conditions primary cells will grow and proliferate, but they are only able to do so a finite number of times (7). This number is known as the Hayflick limit (7). The Hayflick limit is related to the telomere length at the end of the cell’s DNA. As cells undergo each cell division, a small segment of the telomere is lost after each time DNA is duplicated. This process eventually leads to a stage of senescence, where the cells can no longer divide. Continuous cell lines, on the other hand, are able to escape the normal constraints of the cell cycle and grow indefinitely, making them extremely useful for long-term research (8). Whereas primary cells are obtained directly from donor tissues, cell lines can be derived from clinical tumors, or created from transforming primary cells with viral oncogenes or chemical treatments.
Cell Cycle and Immortalization
While primary cells are known for their virtue of retaining most of the characteristics of the original tissue from which they are derived from, they are difficult to maintain in culture and can have batch-to-batch variation. Immortalized cells offer a solution to this setback and present a constant supply of research material.
In order to understand how cells are immortalized, it is first important to understand the mammalian cell cycle. There are 5 phases in the mammalian cell cycle: three gap phases, G0, G1and G2, a synthesis phase and a mitosis phase (Figure 1) (9). These 5 phases can be clumped together into 2 major parts: interphase, which includes the gap phases and synthesis, and mitosis (9). When a cell is in G0, it is simply in a quiescent state and is not preparing to divide. The binding of mitogens, like growth factors, will cause the cells to leave G0 and enter G1, thereby preparing to divide. Mitogens are substances that induce cells to undergo mitosis (10). During G1, S, and G2, the cell is making RNA, various proteins, and duplicating DNA in order to prepare for division, i.e. mitosis (9).
Figure 1 – Cell Cycle
There are several checkpoints in between phases in the cell cycle that ensure that the cell meets the appropriate requirements in order to move on to the next stage. The first checkpoint is at the end of G1. Known as the restriction point, it allows cells to commit to cell division. The second is in S-phase and is there to check the quality of the replicated DNA and to determine if any DNA repair mechanisms need to be activated. The third checkpoint is after G2, and its purpose is, again, to ensure that DNA has been replicated completely and is undamaged. Finally, there are several checkpoints within mitosis to ensure that the cell is in a proper position to complete cell division, i.e. cytokinesis (9).
There are various molecules that are in charge of controlling the stages of the cell cycle, molecules like cyclins, cyclin-dependent kinases (Cdks), and Cdk inhibitors (9). Generally, cyclins bind to Cdks in order to form complexes that are then able to move the cell from one part of the cycle into the next (9). Cyclins are created and destroyed throughout the cell cycle and their levels can help to determine approximately what stage of the cycle the cell is in (9). Cdk inhibitors, as their name suggests, can bind to these cyclin-Cdk complexes and prevent them from initiating the next stage in the cycle (9).
Another protein, the tumor suppressor protein p53, is also vital, both to the cell cycle and immortalizing cells. It comes into play at the checkpoints after G1 and G2; if there is DNA damage, it will induce transcription of the Cdk inhibitor p21, which will prevent the activation of G1 cyclin-Cdk complexes (9). Inhibiting p53 will allow the cell cycle to progress and continue proliferating, and can result in cell immortalization. Some ways to do this are by introducing the E6 and E7 oncogenes or the E1a and E1b proteins, among other possible methods (10)(12).
The E6 and E7 oncogenes from the Human Papillomavirus can degrade the tumor suppressors p53 and pRb, which, as mentioned previously, play crucial roles in preventing the progression of the cell cycle at the G1 and G2 checkpoints (13)(14). E6 can also induce the expression of the hTERT gene, which codes for a telomerase that can prevent the degradation of telomeres at the ends of DNA (14). The E1 proteins from some adenoviruses are also able to bind p53 and have effects similar to those of the E6 and E7 oncogenes (15).
There are many other ways to immortalize cells. One way is to introduce a catalytic version of telomerase into cells to prevent their telomeres from shortening (6)(10)(16). Telomeres shorten over time and it is thought that it is this shortening that leads to aging and cell death (16). Another way is to alter cell cycle checkpoints like inactivating p53 and pRb, both major controllers of the cell cycle (10) (Figure 2).
Figure 2 – p53 and pRb in cell cycle
It is important to note that not all immortalized cells will retain the same characteristics as the primary cells and often times characterization experiments are performed on the newly established cell lines to determine their usefulness in research settings.
Figure 1 – Cell Cycle
There are several checkpoints in between phases in the cell cycle that ensure that the cell meets the appropriate requirements in order to move on to the next stage. The first checkpoint is at the end of G1. Known as the restriction point, it allows cells to commit to cell division. The second is in S-phase and is there to check the quality of the replicated DNA and to determine if any DNA repair mechanisms need to be activated. The third checkpoint is after G2, and its purpose is, again, to ensure that DNA has been replicated completely and is undamaged. Finally, there are several checkpoints within mitosis to ensure that the cell is in a proper position to complete cell division, i.e. cytokinesis (9).